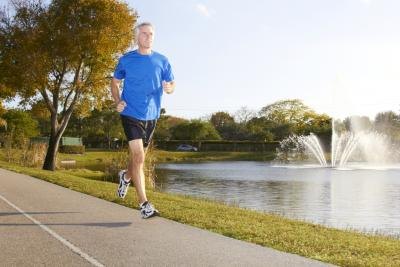
Walking and running are effective cardiovascular exercises that can help you burn calories and improve your health. The Centers for Disease Control and Prevention recommends that adults up to age 65 have several sessions of moderate to vigorous physical activity per week such as aerobic exercise. Both walking and running can help you achieve the goal of including moderate to vigorous physical activity every week depending on the intensity you put into these exercises.
Calories Burned
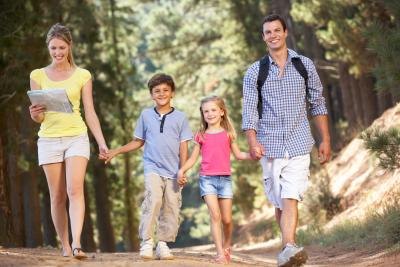
Although there has been much debate about the number of calories burned from walking versus running the same distance, a study in a 2012 "Journal of Strength and Conditioning Research" showed that participants who walked one mile burned 89 calories during the exercise and 110 calories over the next few hours after the workout, while participants who ran one mile burned 112 calories during the exercise and 159 calories as the day progressed.
Joint Impact
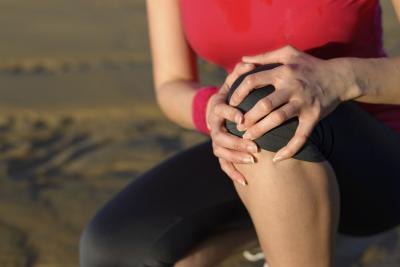
The impact on your joints is generally less from walking than running because of the increased up-and-down movement of your body when running, which increases joint impact. You can work with an exercise science specialist or a physical therapist to improve your running gait and reduce your up-and-down motion. However, bouncing off the ground when you walk can increase the impact on your joints, so take care to walk evenly, with little bouncing.
It's All About Intensity
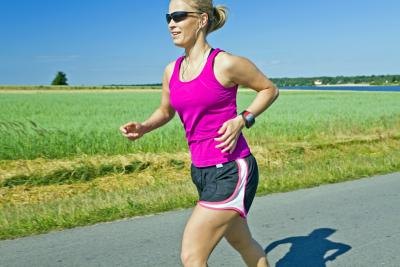
One of the most important factors determining how effective your workout is from walking or running is the intensity of effort. For instance, running on a level path requires less effort than running up a hill, and walking at a brisk pace requires more effort than a leisurely walk does.
www.livestrong.com